Membranes as Therapeutic Targets
The importance of
membranes
Human
Disorders
Cell Membranes
Membranes are an integral part of life defining cellular structures
that have evolved to compartmentalize metabolic activity, protect
cells and organs, and provide means of communication over long and
short distances. The activity of membranes can be broadly classified
as signaling (communication), transport (exchange), and providing
structural coherence and functional independence of cells, tissues,
and organs. In complex organisms like human beings, the function
of membranes is crucial for development, metabolism, motility and
reproduction.
Signaling is important for sensory organs, but also defense (immune
system), and overall metabolic integration (nervous and endocrine
system). Sensation and regulation are of course dependent on enzymatic
reactions and transport of metabolites throughout the body. Absorption,
distribution, energy conversion, and secretion are part of a metabolic
cycle that extracts energy and matter from the environment for the
benefit of a stable, ordered multi-cellular organism. The maintenance
of order based on a steady flow of matter and energy is referred
to as metabolic homeostasis. To keep the trillions of cells in a
stable form, extracellular protein and carbohydrate components are
anchored in cell membranes contributing to cellular adhesion (junctions,
connective tissue) forming a structural framework (scaffold) important
for development, growth, tissue integrity and regeneration.
Membrane proteins contribute to human
disorders by causing faulty regulation, transport, or cellular
integration of tissues. This can be the result of mutations (hereditary
diseases), damaged proteins, or susceptibility to toxins. There
are four distinct ways mutations can affect the proper functioning
of membrane proteins:
1. defective synthesis (transcription, translation)
2. defective processing (folding, assembly, intracellular transport)
3. defective regulation (ligand binding, phosphorylation)
4. defective function
In addition, some cell surface proteins are the target of antibodies
in autoimmune diseases. This leads to the attack and destruction
of the cells and tissue expressing the corresponding proteins. Examples
are Myasthenia Gravis (read
more about it @ eMedicine), a destruction of neuromuscular junctions
targeting the nicotinic acetylcholine receptors, or Pernicious
anemia (read
more about it @ eMedicine), an autoimmune disease of the stomach
lining targeting the H/K-ATPase (pump) in gastric pit epithelia.
The resulting loss of parietal cells means reduced levels of intrinsic
factor, a binding protein of dietary cobalamine, leading to vitamin
B12 deficiency.
Diseases involving membranes are either the result of unregulated
signal transduction pathways or defective transport systems. Antagonists
are the most common drug class targeting membrane proteins that
are integral parts of such pathways. Therapeutic intervention of
antagonists works by blocking ligand binding sites on receptors
or interfering with the reuptake of signaling molecules (e.g. Prozac)
to prolong the effect of a hormone or neurotransmitter. Diseases
related to transporters, however, usually require restoration of
function rather than suppression of an enzyme gone wild. This is
undoubtedly a more challenging approach for drug development, as
rescue of function is not as easily achieved as inhibition of function.
In many cases, replacement of faulty proteins by gene therapy or
protein replacement may be the only solution. In some instances,
however, small peptide mimetics that function as folding templates
for misfolded proteins in the endoplasmatic reticulum (ER) can rescue
defective proteins and support their transport to the cell surface.
An example is IN3 a peptide mimetic that has been shown in vitro
(cell culture) to rescue the cell surface expression of Gonadotropin
releasing hormone receptor (GnRHR), a member of the G-protein coupled
receptor family (P.M.Conn et al., 2002, Mol Intervention 2:308).
Eukaryotic cells have multiple membrane
structures separating compartments with functional specialization.
Membranes serve as gatekeepers of the flow of information and metabolites
between compartments within cells as well as between cells and their
surroundings. In multicellular organisms, adjacent cells attach
by forming junctions that can be permanent and last throughout the
life of an organism, or temporary ones that are forming for specific
periods of time during development, growth, wound-healing, or immune
defense. Cells come in a variety of shapes and metabolic functions,
differing in their structural organization with usually asymmetric
shapes. These differences are the result of distinct temporal expression
and spatial distribution of identical or closely related proteins.
The employment of the same members of protein families in different
cell types explains why hereditary diseases caused by a mutation
of a transporter can show neurological, immunological, and metabolic
defects. Cystic
fibrosis (CF @
eMedicine), a defect in epithelial chloride transport, affects
different organs including the lung, intestine, pancreas, kidney
and sweat glands.
Most membrane bound processes involve the coordinated activity
of several different types of membrane proteins. A well understood
'complex' system is the electrical excitability of membranes. Action
potentials are the result of the orchestrated activity of several
types of ion channels with distinct gating properties and ion selectivities.
An other example of coordinated activity of channels is the control
of insulin release form the pancreas. Glucose influx into beta-cells
leads to ATP dependent K-channel activation. The resulting depolarization
opens a voltage gated Ca-channel. Calcium influx triggers vesicle
fusion and the release of insulin. An autocrine feed back mechanism
via the insulin receptor stimulates additional insulin synthesis
priming the beta-cells for a next round of glucose mediated hormone
release.
It should be noted, that the blood serum concentration of most
metabolites is carefully regulated and maintained within a narrow
physiological range. Besides glucose, the homeostasis of nitrogen
levels is the most important integrative factor in metabolism. Nitrogen
containing compounds are mostly amino acids, with many minor substances
(lipids, nucleic acids, specialized carbohydrates on cell surfaces)
and the body maintains a tight balance between dietary nitrogen
(protein), internal nitrogen that is recycled (tissue protein),
and secretion of nitrogenous compounds (urea, ammonia, uric acid).
The amino acid glutamine is the central metabolite that is regulated
and plays an important role in coupling amino acid metabolism with
that of sugar and fat. Needless to say, many membrane transport
systems are required for absorption, distribution, and secretion
of nitrogenous compounds. Liver and kidney cell membranes play an
important role in this homeostatic mechanism.
To get the right appreciation of these membrane processes, it is
necessary to have a rudimentary understanding of cell membrane structure
and its components, the lipids and proteins. Detailed descriptions
can be found in accompanying lectures
on this site. To briefly summarize, membranes are composed of
bilayers of phospholipid monolayers. Membranes thus form elongated,
flat sheets with a water soluble, charged surface on each side separated
by an internal hydrophobic layer. This hydrophobic layer is made
of fatty acids and cholesterol (the latter found only in animal
membranes) and functions as an electric insulator and barrier against
all water soluble molecules, samll and large. This water soluble
molecules can only cross cell membranes with the help of membrane
proteins that function as transporter, facilitating diffusion or
requiring chemical energy to actively push them across the barrier.
The basic activity of membranes is that of a fluid-mosaic structure
that is fairly stable, yet highly dynamic and promotes lateral diffusion
of its components from one side of a cell to another. Mobility is
a function of size of the molecules - the larger the slower, resulting
in rapid lipid mobility, but slow membrane protein movement. The
latter are often organized in large complexes and clusters reducing
the mobility to near zero, allowing permanent anchoring on the cell
surface. Consequently, immobile membrane proteins can associate
and organize underlying cytoplasmic structures like the cytoskeleton
fiber network. The latter mechanism has enormous impact on the overall
structure and activity of a cell.
Due to the dynamic of its lipids components, membranes can also
fuse together when put right next to each other. Membrane fusion
(and its reverse, membrane vesicle budding) are important cellular
processes. Because of their importance, fusion is not random but
tightly controlled by fusion proteins that gives cells control over
timing and location of fusion. Endo- and exocytosis are the most
important physiological mechanism involving these fusion events.
In the laboratory, entire cells can be tricked into fusing their
cell membranes producing artificial cells and cell hybrids.
The general architecture of membrane proteins provides two distinct
forms; first, transmembrane or integral membrane proteins; second,
peripheral membrane proteins that are anchored by lipid residues
(e.g. G-proteins) or attached by electrostatic interactions (e.g.
mitochondrial cytochrome c). The structures of many membrane proteins
(30+) has been solved since the first such report in 1985 of the
bacterial photosynthetic reaction center. The number of structures,
however, is still very small compared to the number of structures
of water soluble (so called globular) proteins that ranges in the
10,000. The difference is due to the difficulty of handling the
water insoluble membrane proteins under experimental conditions
suitable for structure analysis.
The hydrophobic characteristic and the structural feature of many
membrane proteins (being exposed to the cell surface) makes them
susceptible to toxins and drugs that affect membrane function. General
and local anesthetics are prominent examples of small molecules
that have inhibitory effects on the electrical properties of muscle
and neuronal membranes, thus their ability to numb (local) or render
us unconscious (general). General anesthetics are hydrophobic molecules
of various structure that preferential bind to the lipid portion
of membrane and through some not yet fully understood mechanism
suppress brain activity regarding awareness and pain sensation.
Local anesthetics, however, are water soluble molecules that bind
to certain membrane proteins, called ion channels, interfering with
their ion conduction property. Thus, they suppress action potential
dependent cell function suppressing neuronal signaling of sensation.
Natural toxins have long been used as pharmacological agents to
purify and study channels. Many naturally occurring molecules found
in venom of snakes and spiders have helped us understand the mechanism
of the inhibitory function of drugs. These toxins are potent inhibitors
of channels and pumps or sometimes overstimulate them, causing muscle
cells to stop cycling through contraction and expansion. Proper
dosage can turn toxins into useful modulators of channels and pumps.
Cardiotonic steroids are examples of naturally occurring drugs that
affect the Na/K-pump and thus the baseline ion gradients necessary
for proper action potential activation and duration.
Back
to Top
Signaling disorders
Tyrosine
Kinase Receptors
G-protein coupled receptors
Cell adhesion molecules
Gap Junctions
Electrical Excitability of Membranes
Signaling is important for integration of metabolism and coordination
of organ activity and defense. Sensory organs, the nervous, endocrine,
and immune system make extensive use of signaling processes. An
important aspect of signaling is direct cell-to-cell communication
regulating cell adhesion and migration, differentiation, growth
(development, cancer), cellular immunity and blood clotting. At
the center of signaling processes are cell surface receptors and
receptors on organellar membranes. Some signaling molecules can
diffuse across the hydrophobic membrane due to their low water solubility
properties binding to cytoplasmic and nuclear receptors. Signaling
via cell surface receptor regulates four major physiological processes.
They are:
1. Gene expression activity
2. Metabolism via enzyme modification
3. Cytoskeleton organization / restructuring
4. Membrane permeability
Receptors are integral membrane proteins with large extracellular
and cytoplasmic domains. As is true for any protein system, receptors
have an intrinsic internal flexibility that is exploited to regulate
the activity of cytoplasmic domains in response to a ligand binding
event on their extracellular domains. This process is the canonical
receptor mediated activation of a cytoplasmic signaling cascade
and known as transmembrane signaling. Such cascades are amplifying
a signal where a single ligand-receptor binding event can stimulate
dozens of first responses (activate several G-proteins, phosphorylated
several kinases) which in turn can each activate another dozens
of secondary targets and so on. The result is a single hormone activating
hundreds of enzymes. Mechanistically, an extracellular ligand activates
a integral membrane protein that changes its cytoplasmic activity
through an allosteric mechanism. Allosteric mechanisms are due to
protein conformational changes upon ligand binding, affecting enzyme
activity or secondary ligand binding on the same protein complex.
Models of receptor activation
usually assume a two state model with an active and an inactive
form. In biochemistry, a receptor can switch between both forms
and this process is described as an equilibrium. The important point
is the control over this equilibrium, that is to say, how many receptor
units in a population at any given time are in one or the other
state, active or inactive. Ligand gated receptor are most of the
time in an inactive conformation. For most receptors, this is a
monomeric form, a single copy of a receptor unit. Ligand binding
stimulates dimerization with the dimer being the active conformation.
We say that the equilibrium is shifted from one to the opposite
state. A typical activation scheme is shown for the tyrosine
kinase receptors which are the targets of growth factors and
hormones.
Dimerization of the extracellular domains results in dimerization
of a kinase domain on the cytoplasmic side of the membrane causing
autophosphorylation of tyrosine residues. Phosphorylated receptor
dimers will bind target proteins and phosphorylate starting a cytoplasmic
signaling cascade. The phosphorylated domains recognize adapter
modules on target proteins. These adapter modules are either
SH2 domains which bind Tyr~P, or SH3 domains which bind proline
rich domains. Binding between kinase and target protein brings the
latter into contact with the active site of the kinase leading to
phosphorylation of the target proteins. An other known adapter module
is the PH domain (Pleckstrin homology) which mediates membrane contact
through binding to phosphorylated phosphatidylinositols.
Dimerization is not necessarily an activation control mechanism.
In protein tyrosine phosphatases (PTPs) the monomeric cell
surface molecule is the active conformation resulting in the dephosphorylation
of P~tyrosine residues. Dimerization of PTPs leads to their inactivation.
It can be hypothesized that PTP monomers can disrupt dimers of tyrosine
kinase receptors. Consequently, phosphorylation is reduced, while
dephosphorylation activity is increased. Down regulating the number
of PTPs will allow increased kinase dimers (causing overall signaling),
while down regulating kinase receptors will cause PTP dimers (causing
overall signal inhibition).
An another example where dimerization plays
a role in controlling signaling are the G-protein coupled receptors
(GPCRs). Here, monomers are mostly the active form, although some
types (e.g. GABA receptors) are found to form functional heterodimers.
GABA(B1a)-GABA(B2) heterodimerization has been shown to be necessary
for cell surface expression. In other cases, dimerization leads
to different ligand specificity or as compared to the monomeric
conformation. For the beta-adrenergic receptors, monomers are the
active form and dimerization has been associated with inactivation
by recycling the receptor or removing them permanently from the
cell surface through endosome mediated degradation..
Due to the lack of high resolution structures of ligand gated receptors,
the details of allosteric activation are not fully resolved. Some
enzyme systems, however, have shed light on the model of allosteric
regulation. The positive cooperativity of oxygen binding to
hemoglobin tetramers is an example thanks to the availability of
crystal structures of both a completely oxygen free (T-state) and
a fully oxygen saturated structure (R-state). Differences in subunit
organization (quaternary structure) and back bone folds in each
subunit at both the heme (ligand binding site) and subunit contact
sites largely explain the conformational change and the stability
of both R and T-state tetramer conformations. An other example is
the ras GTPase (related to the alpha subunits of G-proteins)
with a GTP (active) and GDP bound (inactive) high resolution structure.
G-protein coupled receptors form a large and diverse group of membrane
proteins that share a simple seven transmembrane helix motif. The
structure of this membrane domain has been solved from X-ray crystallography
of rhodopsins - both the distantly related bacterial proton pump
bacteriorhodopsin, and the light sensitive rhodopsin from bovine
retinal cone cells. No structure of a related ligand binding GPCR
is available. In the absence of high resolution data, but availability
of sequences and close similarities in membrane spanning domain
architecture, modeling of many receptors based on the backbone conformation
of bovine rhodopsin helps understand ligand binding diversity of
the G-protein coupled receptor system. Extracellular and intracellular
loops are the primary binding domains, with the transmembrane helices
also serving as binding cavity for small ligands such as acetylcholine,
dopamine, glutamate, epinephrine (adrenaline), or serotonine.
Approaches of modeling related receptors based on the availability
of structures from a member of a protein family is an important
novel strategy in post-genomic analysis of biological systems.
Thanks to the domain module architecture of proteins, and the conservation
of domains in proteins with diverse function (e.g. immunoglobulin
domains in tyrosine kinase receptors), the structural conservation
can be exploited to predict structures or functional association
to novel gene sequences based solely on patterns of similarity in
databases. Similarity can be based on sequence similarity (classic
BLAST algorithms), similarity in motif, domain, or tertiary structure
(proteomics and structural genomics), or similarity in genomic organization
(comparative genomics) and co-expression with related genes that
form part of a pathway (functional genomics, metabolomics).
Many diseases are related to lack of binding, surface expression,
or G-protein coupling of these receptors. Diseases can also be related
to malfunctioning G-proteins and some bacterial toxins are known
to overstimulate G-alpha subunits through covalent modification
and constitute activation of effector activity (cAMP production).
Cholera toxin stimulates Gas type subunits causing diarrhea
and pertussis toxin activates inhibitory Gai subunits, a
major cause of whooping cough. The specificity of these toxins for
either alpha type has been instrumental in analyzing the effector
diversity of G-proteins and comprises an excellent example of biochemical
analysis of parts of a signal transduction pathway. An example of
GPCR linked disease is congenital nephrogenic
diabetes insipidus, where kidney loose response to vasopressin
or antidiuretic hormone (ADH). Here, the vasopressin 2 receptor
(VR2) can be inactive when mutations cause a it to loose hormone
binding, G-protein coupling, or surface expression. The latter can
be classified as a misfolding disease where the receptor
is most likely stuck in the ER membrane. Some examples of rescue
therapies for misfolded proteins have been reported where small
molecule templates such as peptide mimetics can bind to the newly
synthesized protein and assist it in proper folding.
With intense scrutiny of a system often come novel insights. G-protein
coupled receptors have been identified to exhibit activities that
do not include G-proteins, but depend on the presence of oligomerization
domains. The domains are usually short, highly conserved amino acid
sequences that mediate specific interactions between proteins resulting
in large complex formation. Many membrane associated signaling
complexes are know being studied. One example is the post-synaptic
organization of glutamate regulated long term potentiation. Here,
metabotropic and ionotropic glutamate receptors are coupled to a
mediator protein (shank/proSAP) which contains multiple interaction
domains, including a PDZ domain, Homer, Ankyrin, and an SH3 domain.
As a mediator, this protein organized a membrane bound cluster connecting
the cell surface with cytoskeletal actin filaments.
Homer domains have been identified by studying the coupling of
metabotropic glutamate receptors with the calcium release channel
of the endoplasmatic reticulum. Here, homer protein dimers mediate
the direct protein-protein interaction between the cytoplamsic and
ER membrane by binding to both the mGluR and Ryanodine receptor.
A member of the homer family that is unable to dimerize can be upregulated
and competes with homer dimers, thus counteracting the plasma membrane
to ER membrane coupling. The function of PDZ domain has been shown
for beta-adrenergic receptors that bind via a C-terminal DSLL motif
to a PDZ domain of the sodium-proton-exchanger regulatory factor
(NHERF) providing a G-protein independent control of electrolyte
homeostasis by epinephrine.
In yet another G-protein independent pathway, beta-arrestin has
been implicated in controlling localization of signaling complexes
rather than just receptor desensitization. Beta-arrestin is
involved in the regulation of c-Src family tyrosine kinase activity
in response to chemokine stimulated degranulation in neutrophils.
Beta-arrestin controls a complex formation of chemokine receptor
with a tyrosine kinase receptor and through vesicle mediated trafficking
of CXCR1 (a G-protein coupled chemokine receptor) directs the co-localization
of tyrosine kinase receptors with granule vesicles. Such a coupling
of GPCR internalization and activation of tyrosine kinase receptors
can be viewed at as the timing of neutrophil activation where chemokine
sensitivity is inactivated and degranulation activated making neutrophil
activation irreversible.
Signaling is often understood as the result
of a diffusible ligand activating its receptor. However, a no less
important form of receptor signaling involves the use of non-diffusible
ligands. Unlike neurotransmitters, hormones, and growth factors,
a diverse group of cell surface molecules recognize other cell surface
molecules. Either homotypic or heterotypic recognition is possible.
The resulting cell adhesion and formation of various types of permanent
and temporary junctional complexes is important for development
and differentiation. Tight junctions, desmosomes, and gap junctions
provide both tissue integration as well as cell to cell communication.
Temporary junctional complexes have recently been described between
T-cells and antigen presenting cells (APCs) forming immunological
synapses that regulate T-cell maturation. Lack of proper cell adhesion
can cause cancer growth and metastasis, a process of cell migration
with subsequent binding and proliferation of cancerous tissue.
Four major classes of cell adhesion molecules have been
characterized. They are:
1. Cell adhesion molecules (CAMs)
2. Immunoglobulin like cell adhesion molecules (Ig-CAMs)
3. Integrins
4. Selectins
Cell adhesion molecules or CAMs are mostly expressed in
cell type specific patterns and regulate homotypic cell recognition
in a calcium dependent manner. Lack of these CAMs results in loss
of cell contacts and potentially cancer and their presence is guiding
adjacent cells to form stable contacts and differentiate into tissue
specific cells. The best known type is E-cadherin that is specifically
expressed to mediate homotypic association between cells, particularly
epithelial cell layers (hence the E). E-cadherin has first been
shown as uvomorulin to control morula compaction in early development.
The major location of uvomorulin (E-cadherin) is the intermediate
junction in the tripartite structure between adjacent cells
in epithelia. The three junctions found there are the tight junction
towards the apical membrane (e.g. lumen of the intestine), followed
by the intermediate junction and the desmosome structure located
toward the basolateral side of the epithelia. Cadherins form intermediate
junctions by inducing a zipper like complex between multiple
E-cadherin dimers on each cell surface. Cadherin clustering is followed
by intermediate filament assembly (alpha and beta-actinin, plakoglobin)
and actin skeleton organization.
Ig-CAMs are more diverse and function in a calcium independent
fashion. They also can mediate homotypic as well as heterotypic
cell adhesion. Splice variants of Ig-CAMs have been described that
provide different ligand specificity and different cell membrane
anchoring. A shift from transmembrane to GPI anchor (an extra cellular
lipid anchor of cell surface proteins) allows CAM binding domains
to rapidly diffuse along cell surfaces or to be released from its
anchor through phospholipase mediated hydrolysis. Thus, cells can
rapidly switch from adhesion to loss of adhesion or provide soluble
ligands that can interfere with neighboring cell adhesion events.
Integrins are a heterogeneous class of cell adhesion molecules
that mediate signaling between extracellular matrix (ECM) components
and the cytoskeleton. Integrins operate as heterodimers of alpha
and beta subunits. From a selection of 19 alpha and 8 beta subunits,
24 functional alpha-beta dimers have been described so far. The
diversity of integrin dimers is related to diversity of function
that goes beyond ECM interaction. Integrins can also mediate heterotypic
cell adhesion and are involved in dynamic processes that do not
need long time contacts. Integrins control signaling pathways of
the ERK, MAP kinases affecting gene expression needed for differentiation,
proliferation and migration of cells. They integrin mediated ERK
pathway can interact with GPCR coupled ERK signaling demonstrating
cross-talk between cell surface receptors. Integrins also signal
via Rho GTPases directly to the cystoskeleton regulating stress
fiber growth, and the growth of microfilaments in filapodia and
lamellipodia. Filopodia are required as sensory structures and effectors
of motility. They receive signals from the molecular environment
and transmit signals that alter the cytoskeleton and cellular motility.
Selectins are specialized cell adhesion molecules involved
in platelet and leukocyte adhesion to endothelial cells. They play
an important role in recruiting cells in wound healing and inflammation.
Their ligands are carbohydrate rich cell surface proteins (glucans)
or glycolipids. Transient bond formations between the selectins
and their ligands mediate the early steps of the adhesion cascade.
Selectins contain an N-terminal extracellular domain with structural
homology to calcium-dependent lectins such as mannose binding protein.
The latter is part of the innate immune system providing broad protection
against pathogenic microorganisms by recognizing their glycosylated
surfaces. Selectin expression on epithelial cells causes a a slow
movement of leukocytes along the endothelium via transient, reversible,
adhesive interactions. Three types of selectins have been described;
L-selectin is leukocyte specific, P-selectin is expressed on platelet
surfaces, and E-selectin is expressed on cytokine activated endothelial
cells.
Selectins, integrins, and Ig-CAMs are involved in leukocyte
rolling, a dynamic process of cell adhesion mediated between
leukocytes and infected endothelial cells. More about the process
of inflammation
by Klaus Ley and Adam Brooke can be found on-line at the Department
of Biomedical Engineering at the University of Virginia.
The immunological synapse between T-cells and antigen presenting
cells (APCs) is another dynamic system that involves formation of
large protein clusters with functional domain organization. This
'synapse' which resembles more a junctional complex involves many
different types of cell surface receptors including integrins, Ca-independent
Ig-CAMs, and T-cell receptors that form functional contact with
MHC-antigen complexes. Synapse formation through receptor clustering
occurs within minutes resulting in calcium mediated intracellular
signaling in the T-cell, which will be loosing contact and now being
activated for proliferation and differentiation.
Therapeutic strategies involving cell adhesion molecules:
- Regeneration of axons of damaged nerve tissue by providing
exogenous CAMs and growth promoting adhesive molecules like
laminin, collagen and fibronectin (receptors for integrins)
- Augmentation of cancer metastasis, inflammation, and thrombosis
by inhibiting CAM function (e.g. antibodies to lung specific
epithelial CAM (Lu-ECAM-1) produced 90% reduction in integrin
mediated tumor cell adhesion)
- CAM modulation in autoimmune disorders and transplant
rejection. Corticosteroids as anti-inflammatory agents suppress
induction of E-selectin and ICAM-1 preventing recruitment of leukocytes
(see also previous slide).
- Antiviral therapy through prevention of pathogen-cell interaction
by soluble analogues of Ig-CAMs; e.g. ICAM for rhinovirus
(common cold); CD4 for HIV)
Dual function proteins have been described that have both cell
adhesion domains and a transmembrane domain with transport function.
An interesting example is the mechanosensitive cation channel involved
in adult polycystic
kidney disease or APKD. Another is Lu-ECAM-1 which has also
been described as a calcium activated chloride channel (CaCL). Both
protein systems show sensitivity towards proteases and separation
of the extracellular cell adhesion domain from the channel domain
appears to be an activation mechanism in the transport function
of these dual function proteins.
A specialized junctional complex between
adjacent cells is that formed by connexins or gap junction
proteins. Gap junction channels cluster into fairly large 'plaques'
that can contain thousands of individual channel units. Gap junctions
provide metabolic coupling allowing the simple diffusion
of small metabolites between cells without them being transported
out of cells and back into them. Because gap junction channels are
constitutively open (and can be closed by voltage, local anesthetics
or lack of calcium) they also provide electrical coupling
between neighboring cell membranes. Metabolic coupling can occur
over several cells forming metabolic supercompartments called a
syncytium (a polynucleated cytoplasm). Similarly, electrical coupling
has been shown to coordinate action potential activity in muscle
tissue of entire muscle fibers to promote synchronous calcium triggered
contraction-relaxation of muscle.
Structure
of gap junction channel
Gap junction
channels are made of two hexameric units, each of which forms
a membrane spanning channel (see phospholipids to the left
for membrane location). Two such channels (dubbed hemi-channels
or connexons) interact with their extracellular domains connecting
the two membranes and forming a continuous cytoplasmic connection
between the two cells. Subunits have four helical transmembrane
spanning segments with segment M3 facing the channel wall
(dashed cylinder in upper hemi-channel). The pore is fairly
large estimated to have a 2nm diameter and permeable to second
messenger molecules like calcium, cAMP, IP3, but not peptide
and proteins. Exclusion size limit is about 1kDa. Internal
charges near extra-cellular gap provide some charge selectivity
to ionic species that varies with connexin type.
|
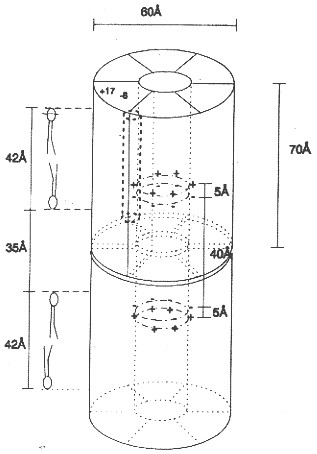
|
Although gap junction structure and function is fairly well characterized
by genetic and cell biological studies and heterologous expression
systems, their physiological role eludes clear understanding. In
vertebrates some 17 different connexin genes have been identified.
Connexins are expressed in every cell type, except single celled
erythrocytes. Every cell studied co-expresses at least two types,
although their functional and structural differences are not drastic.
Knockout studies indeed demonstrate that despite the cell type specific
expression, no lethal phenotype arises indicating a strong redundancy
in gap junction function. The most interesting aspect of gap junction
coupling is the diffusion and local distribution of second messenger
molecules. All gap junction channels so far tested show some selectivity,
but are not highly specific and thus their commonly large pore makes
them equally permeable to nucleotides, sugars, and amino acids and
their derivatives.
Many diseases related to connexin mutations have been characterized.
For example, some mutations (but not others) in connexin 26 (also
known as beta2) have been linked
to deafness. Interestingly, while these mutation cause disorders
in the peripheral nervous system, they do not affect liver function,
the organ that strongly depends on the expression of connexin 26.
Gap junctions of the inner ear may play a role in maintaining potassium
homeostasis, which is important for inner-ear function and, thus,
hearing. It has been proposed that mutations in Cx26 may disrupt
potassium circulation and result in deafness. A peripheral nervous
system disease linked to the other liver resident connexin 32 is
called X-linked Charcot-Marie-Tooth
(CMTX) disease. CMTX is a group of hereditary motor and sensory
peripheral neuropathies caused by demyelinating axons of peripheral
neurons. Again, connexin 32 mutations do not affect liver function.
Deafness is a complex dysfunction and many different proteins have
been identified in different forms of hearing loss or impairment.
This includes transcription factors, potassium channels, extracellular
matrix proteins, cadherins, tyrosine kinase receptors, an ABC transporter,
serine proteases, and an anion transporters. The latter has been
found to cause Pendred
Syndrome, a hearing loss due to insufficient supply of sulfate
ions for bone formation in the cochlea. The transporter, who has
been well characterized and is highly expressed in the thyroid where
its role is to facilitate iodide uptake, is also permeable for chloride
and sulfate ions.
Electrical excitability
of neuronal and muscle cell membranes allows the rapid transport
of information along cell surfaces. This form of lateral signaling
is manifest in periodic action potentials leading to cell type specific
'firing' patterns. Chemical and electrical synapses,
in turn, transmit the electrical signal across inter-cellular gaps
to neighboring cells. Proteins involved in electrical signaling
are ion channels and pumps. The former mediate the signal, while
the latter restore the used-up ion gradients. Mutation in channels
can cause arrhythmias in heart muscle due to changes in channel
gating, ion conduction, or abolished function. Many drugs bind to
channels and pumps affecting the periodicity of signaling or open
times of channels and pumps.
Action potentials can be understood in terms of tightly
regulated ion channel activity. Action potentials are temporal changes
in membrane potentials. At rest, cell membrane have a voltage around
-40 to -80mV, meaning the inside of the membrane has fewer positive
charges than are found near the outside surface. Voltage changes
can be triggered by opening ion channels. Usually, a ligand gated
channel (or mechanosensitive channel) opens a cation selective pore
facilitating Na+ influx bring more positive charges into the cell
(inward currents). The resulting change in potential from inside
negative to more positive values is called depolarization and causes
subsequent activation (opening) of voltage gated Na-channels bringing
even more positive charges into the cell. Next, voltage gated K-
and Ca-channels open. The K-channels allow K+ efflux reversing the
depolarizing effect of Na influx bringing the potential back to
negative resting values. Calcium channels, however, reinforce influx
of positive charges (Ca++) and their effect is to modulate the length
of the action potential slowing down hyperpolarization by K+ efflux.
Importantly, all channels will spontaneously inactivate with varying
kinetics. Thus, cell type specific expression of channels with different
activation and inactivation kinetics will produce different 'shapes'
of action potential, some with rapid increase and decrease, some
with slow increase and/or slow decrease. The length and profile
of action potential is tuned to physiological needs and any deviation
from these 'firing' patterns can cause malfunction of neuronal coupling
or the repetitive pattern of muscle contraction - relaxation cycles
such as in heart. Many arrhythmias have been traced to mutations
in voltage gated channels that alter the length (or period) of the
action potentials controlling heart beat. The long
QT-syndrome is one of the physiologically best understood hereditary
disease underlying heart arrhythmias.
For historic reasons, the ion channels of excitable membranes responsible
for action potentials have been studied extensively by electrophysiological
and pharmacological means. Most of the channels directly involved
in electrical signaling are cation selective. However, the existence
and involvement of cation selective channels is an important aspect
in understanding inhibitory and hyperpolarizing effects on
neurons and muscle cells. In other words, chloride channels are
well known to suppress electrical activity of excitable membranes.
They control the frequency and length of resting periods in neurons
and their defects can cause malfunctioning and overstimulation of
neuronal activity. Mutations in chloride channels have been linked
to diseases. The focus here will be on myotonias and myopathies,
muscle anomalies and weakness caused by chloride channel
mutations. Other diseases are cystic fibroses, anxiety, renal failure,
kidney stone disease, neuropathic pain, and neuronal and liver encephalopathies.
Like any other channel system, chloride channels form a large and
structurally heterogeneous group with ligand gated (glycine, GABA
gated channels in inhibitory synapses) and voltage gated types (ClCs),
but include also CFTR, an ATP coupled transporter (see cystic fibrosis).
The structure of a bacterial voltage gated chloride channel
has been solved. It is a dimer with two channels that can open and
close independently but also in coordination. The involvement of
alpha helical dipole structures to stabilize permeant ions has confirmed
the use of such motifs in ion selectivity in general. The tunneling
of ions across membranes can also be compared to substrate tunneling
in enzyme complexes that provide efficient throughput of metabolic
intermediates in a pathway. A voltage gated chloride channel unrelated
to the ClC family and also sensitive to calcium regulation has been
identified in epithelial cells on the apical surface. This channel
is a dual function protein with a large N-terminal cell adhesion
domain, which has been independently identified as Lu-ECAM-1, and
Ig related cell adhesion molecule. Expression, molecular weight,
and post-translational hydrolysis are all consistent with the dual
function of this apical membrane protein.
As mentioned above, chloride channels are involved in hereditary
diseases of skeletal muscle dysfunction. But many of these
diseases have several phenotypes, as different ion channels are
involved in electrical excitability. Also the reason for lack of
excitability or overstimulation can vary as proteins may have altered
activation-inactivation kinetics, ion permeability, or defective
cell surface expression. Myotonia
Congenita or Thompson's disease is related to a defect in ClC1,
but also Na-channels mutations have been identified. An example
is Hyperkalemic
periodic paralysis (HYPP) where Na-channel mutation give rise
to cold induced muscle weakness. Malignant
hyperthermia is due to a defect in calcium regulation at the
level of the calcium release channel (ryanodine receptor, RYR1).
If the calcium release channel stays open longer in the presence
of anesthetics, the elevated cytoplasmic calcium levels could result
in permanent contraction causing muscle rigidity and paralysis.
As the cells try to restore normal calcium levels, they are metabolically
active to produce the necessary ATP for the calcium pump. This increased
metabolic activity releases heat, excess lactic acid and carbon
dioxide.
Other myopathies are the result of muscle degeneration or
degeneration of synapses between neurons and neuromuscular junctions.
Defects in ligand gated receptors that are found in large numbers
on synaptic membranes (both pre and post-synaptic cleft) and antibodies
generated against those receptors can cause decreased electrical
excitability or destruction of synaptic membranes. Myasthenic syndromes
are a group of disorders related to many different proteins responsible
for cholinergic transmission in chemical synapses; the acetylcholine
mediated stimulus of action potentials. Affected proteins include
acetylcholine esterase, the nicotinic acetylcholine receptor, but
also Na-and Ca-channels colocalized in the endplate membrane of
neuronal junctions. Myasthenia
Gravis (MG) is due to a reduction in the number of acetylcholine
receptors (AChR) on the postsynaptic membrane. Interestingly, many
patients with MG have an epsilon subunit expressed which is fetal
tissue specific and replaced in adult by a delta subunit. Auto-antibodies
against the epsilon subunit may be responsible for the generation
of MG.
As these degenerations develop over longer periods of times and
only partially affect the tissue, the related syndromes can be medicated
by adding more signaling molecules. This can be achieved by inhibiting
acetylcholine esterase resulting in prolonged presence of acetylcholine
in affected synapses. Other neurotransmitters may experience elevated
concentration by inhibiting their reuptake mechanism.
Back
to Top
Transport disorders
Classification
of transporters
Osmotic processes
Water channels
Intestinal
absorption and secretion
Pumps
or ATPases
Multi-drug
resistance
Transport processes designate the flow of molecules and
ions across cell membranes. Transport processes are regulated and
specific and the result of flux-coupling and synergistic interaction
among several transporters. Transport disorders constitute the largest
class of membrane associated disorders as they relate to absorption,
secretion, homeostasis of metabolite distribution (including water
and salt), and energy conversion. The striking compartmentalization
produced by cell and organellar membranes makes the need for transport
of molecules between those compartments and thus across membranes
obvious. Compartmentalization is also a way of controlling metabolic
processes by controlling substrate availability and sequestration.
Transport proteins carrying ions contribute to signaling processes
as well (see ion channels above) and it is not possible to make
a clear distinction of where one process begins and the other ends.
Transport disorders are caused by defective or unregulated carriers,
facilitators, and pumps. Because of these multifactorial systems
a defect in any protein involved in a specific physiological process
may hamper it. And as many transporters are involved in different
transport processes when paired with different partners, a defect
in a single transporter may affect more than one type of physiological
mechanism.
Defective transport not only results in malabsorption, but
also accumulation of metabolic intermediates, often with toxic consequences.
Other defects play a role in energy coupling (mitochondrial and
peroxisomal disorders) or cell volume regulation. Control of water
flow is important for solubilization and distribution of metabolites,
body temperature regulation through sweating, and waste secretion.
Many bacterial toxins negatively affect a cell's ability to regulate
water and ion absorption and retention causing loss of water (diarrhea)
or the accumulation of metabolites.
Transporters are classified
according to structural and functional categories to highlight their
evolutionary relationship. The two major functional classes are
channels and carriers. Channels are distinguished from carriers
based on flux rate, with channels having a much higher throughput
rate than carriers, which are slower and either depend on energy
to pump or facilitate the exchange of larger molecules across cell
membranes. Channel proteins are categorized into alpha helical proteins,
beta barrel proteins, toxins, and peptide channels. Most channels
and those discussed for signaling functions belong to the alpha
helical channel protein category. Most toxin channels and peptide
channels are also adopting alpha helical motifs. However, they are
distinguished from protein channels because they lack regulation
and are used for defensive purposes resulting in the death of cells
whose membranes they can insert to. Beta barrel protein channels
constitute a minor class of channels called porins with largely
size selective function and some substrate specificity. They are
found in the outer membranes of Gram-negative bacteria. A distant
relative is found in the outer membrane of mitochondria (of bacterial
origin) which serves as a electrostatic screening of metabolites
entering and leaving the mitochondrial compartment. The mitochondrial
porin is also known as VDAC, or voltage dependent anion channel,
with a cutoff limit around 1kD. Carriers are a more diverse
group including primary active transporters (pumps, e.g. Na/K-ATPase),
secondary active transporters (symporters and antiporters, e.g.
Na/glucose-symporter, SGLCT2), facilitators (uniporters, e.g. Glucose
transporter, GLUT2), and group translocators (e.g. phosphotransferase
system, PTS).
Nomenclature
of ion channels found in non-excitable epithelial cells |
CRAC
- capacitative calcium entry channel, Ca release activated channel;
DAC - depletion activated channel
SOC - store operated channel
TRP, TRPL - transient receptor potential
NSC - non-selective cation channel
SMOC - second-messenger operated channel
ROC - receptor operated channel (GPCR controlled)
Kir - K-channel inward rectifier
Kv - K-channel voltage gated and Ca depended, slow activating |
KATP
- K channel ATP regulated
ClCa - calcium activated chloride channel
BCl - big conductance chloride channel
Clvol - volume controlled chloride channel
CLC - voltage gated chloride channel
BKCa - big conductance K-channel
MKCa - intermediate conductance
SKCa - small conductance K-channel
SAC - stretch activated channel
MS - mechanosensitive channel
|
Volume control and concomitant
electrolyte homeostasis and metabolite coupled transport across
epithelial cells is a major function of transporters in non-excitable
membranes. While 'excitability' of membranes refers to the ability
to generate action potentials, voltage sensitivity is important,
too, in non-excitable membranes, as localized de- and hyperpolarizations
are used to control calcium entry and thus exocytosis (e.g. insulin
release from pancreatic beta cells) and other signaling processes.
Besides calcium channels, chloride channels play a major role in
controlling basic electrolyte homeostasis as they allow flow
of the major anion to couple ion flux based on charge neutrality.
The basic mechanism of volume control is the osmotic property of
semipermable membranes that allow the flow of solvent, but
not solutes. Thus, unequal solute concentrations in compartments
separated by cell membranes result in the osmotic flow of water
to equilibrate the chemical potential difference of the two solutions.
Water will generally diffuse into the compartment with the higher
solute concentration and its volume will shrink accordingly. The
compartment gaining water will experience a volume increase. Cellular
compartments with varying electrolyte concentrations and mixtures
of salts and solutes can adjust osmotically driven flow of both
water and solutes by opening and closing water and solute selected
transport proteins. Cells can perform transport processes of various
kinds through their ability to control and adjust basic solute permeability
by regulating gene expression, vesicle trafficking and function
of transporters. Transporters increase the rate of diffusion across
membranes by several order of magnitudes (channels have the highest
increase) and can be kinetically characterized using theories developed
for enzyme kinetics. The permeability rates of solvent and solutes
across lipid bilayers differs considerably. Although water is a
polar molecule, it has one of the highest diffusion rates across
membranes in the absence of transporters (permeability coefficient
~ 3x10-3 cm/sec), while polar metabolites like urea and ions have
permeability coefficients several order of magnitudes lower (urea
~10-6cm/sec; Na+ ~10-14cm/sec).
The recently described water channel
family (aquaporins)
plays an important role in epithelial transport of solutes in the
intestine, kidney, lung, and sweat glands that all need an elevated
water flow across these cell barriers to maintain a steady absorption
or secretion of polar and charged solutes and to maintain osmotic
stability during the process. Aquaporins are water selective channels
(some with selectivity for glycerol, an important metabolite linking
carbohydrate with phospholipid and fat metabolism) that prohibit
the flow of small ions. Aquaporins increase water flow dramatically
above background permeability. Measured by the activation energy
(Ea) and hydraulic permeability coefficient (Lp), expressing aquaporins
in cell membranes decreases the activation energy for water flow
by a factor of 3 to 4, while increasing the hydraulic permeability
coefficient by a factor of about 20. Vesicle swelling assays with
aquaporins have shown the size selectivity and amphipathic property
of solute-channel interaction found in the high resolution structure
of a bacterial glycerol facilitator (GlpF). Glycerol units, although
polar, can adopt an amphipathic structure that allows the solute
to optimally interact with an amphipathic channel wall made up of
polar residues on one side and non-polar residues on the opposite
side of the pore. Nephrogenic diabetes insipidus is the result of
defective water reabsorption in the distal duct tubules of the kidney.
The disorder can be due to defective aquaporins or lack of regulation
of aquaporin expression, under the control of ADH (vasopressin or
antidiuretic hormone, see above GPCR).
Transport across cell membranes is an
important physiological process for the distribution of metabolites
and biosynthetic building blocks, but also waste products and secretion
of excess material. Many diseases are related to malfunctioning
of absorption and secretion or its misregulation causing
systemic imbalances leading to deficiencies or toxic accumulation
of chemicals. Absorption and secretion in the gut and kidney
can best represent the fundamentals of transport mechanisms and
their physiological integration and control. The stomach, small
intestine, and colon form a segmented organ responsible for the
degradation and absorption of nutrients and the secretion of waste
products. Different segments have different absorptive mechanisms.
The small intestine specializes in absorption of water, amino acids,
carbohydrates, fats, vitamins, minerals and drugs. The colon which
is rich in bacteria absorbs water, minerals, short chain fatty acids
(bacterial products), and drugs. An important function of the colon
is to control intestinal fluid balance with the ileum being highly
leaky and the distal colon tight. Leakiness is a function of paracellular
pathway controlled by tight junctions, particularly the zona ocludens
(ZO) controlling epithelial resistance. Tightening of the ZO gives
the control over water flow to the epithelial cells and regulated
transport systems. The result of increasing tightness of the epithelial
mucosa from the ileum to the distal colon is that only a fraction
of the water needed to solubilize metabolites and facilitate transport
is lost through fecal excretion. Up to seven liters of internal
water as part of the entero-hepathic circulation are recycled by
the small intestine (secretion and absorption). An additional 1.5
liter of dietary fluids are taken up with only a fraction (0.1 liter)
lost in the feces. Extra fluid absorbed by the intestine is excreted
in the urine or lost through sweating.
The structure of the intestine provides control over uptake
and secretion and the surface architecture is optimized to increase
the area through multiple folding of the cell layer into villi and
cell membranes into microvilli. The average intestinal membrane
surface area is estimated at about 300m2. The mucosal cell layer
itself is surrounded by circular smooth muscle, connective tissue,
blood vessels, lymph, and supporting ligament. These functional
specialization of the gut allows physiological regulation
of absorption and secretion through neuronal, hormonal, immunological
and lumenal signaling molecules. Neuronal regulation of the gut
by the Enteric Nervous System (ENS) uses adrenergic pathways
controlling absorption and cholinergic pathways controlling secretion.
Together, they mediate the interaction between the gut and the brain
with sensory neurons, interneurons, and motoneurons (controlling
smooth muscle for gut contractions and blood flow) explaining some
of the emotional connections between the brain and digestion. The
villi structure of the epithelial cell layer provides a functional
segregation into absorptive and secretive processes on the tip and
crypt of the villi, respectively. The enterocytes on the villi tip
are specialized in sodium and/or proton driven nutrient uptake,
a classical mechanism of flux-coupling of one solute to the gradient
of another one. Both sugars and amino acids depend on the sodium/proton
driven uptake into the blood stream. Specialized symporters (Na-glucose
SGLT1) on the apical membrane (the intestinal lumen surface) absorb
metabolites which accumulate in the enterocyte and diffuse into
the blood capillaries through facilitators (e.g. glucose transporter,
GLUT2). Secretion of chloride and/or bicarbonate maintains osmotic
homeostasis.
Defects in transport function or its regulation can severally affect
homeostasis and causes excessive water retention or loss. Diarrhea
is one of the most common disease and caused by many different factors
related to neuronal, immunological, hormonal regulation, or lumenal
content, particularly bacterial toxins. Well known (but not necessarily
well understood in terms of mechanism) causes of diarrhea are Cholera,
Inflammatory
Bowel Syndrome (Crohn's Disease), Celiac
disease (also known as celiac sprue and gluten-sensitive enteropathy),
lactose intolerance, and AIDS related diarrhea. While cholera and
AIDS related diarrhea are related to bacterial infections and release
of toxins, Crohn's and celiac disease are the result of damaged
mucosal epithelia due to overractive immune responses and altered
cell adhesion mechanisms. Lactose intolerance and the related glucose-galactose
malabsorption result in accumulation of mono- and disaccharides
in the intestinal lumen and exert osmotic pressure on the epithelia
resulting in the loss of water. Glucose-galactose
malabsorption is well understood at a mechanistic level. Here,
the apical Na-coupled transporter SGLT1 is defective and cannot
be transported to the cell surface. In a functional system, glucose
is transported into the enterocyte along with a sodium ion. Glucose
then diffuses into the portal blood via the glucose-specific transporter
GLUT2. Sodium leaves the enterocyte via the Na/K pump on the basal
membrane and potassium is recycled through a K-channel. This energy
driven uptake of glucose and sodium serves as a prototype mechanism
of absorption and secretion.
Many transport defects affecting the gut also affect other organs
that rely on epithelial absorption and secretion mechanism. This
includes the kidney, sweat glands, and lungs.
Renal
glucosuria is a condition with enhanced glucose in the urine.
The cause is defective reabsorption of glucose from filtered blood
serum fluid in the nephrons of the kidney. The defect is related
to the sodium coupled glucose transporter. Patients with glucosuria
have no intestinal glucose uptake defect, indicating that a different
transporter, SGLT2, is expressed in the kidney epithelia.
A large number of other diseases are related to changes in transport
capacity and specificity. Many of them are clinically well known,
although a mechanism and thus cure eludes scientists. A good example
is hereditary
hemochromatosis. This disease is characterized by an increased
iron uptake across the the intestinal epithelia and subsequent accumulation
of iron in liver and kidney, having toxic effects on these organs.
Different proteins have been isolated with mutations contributing
to the disease. This includes iron transporters (divalent metal
ion transporter, DMT1), iron transport protein receptors (transferrin
receptor, TfR1 and 2), and HFE, an immune system protein closely
related to the MHC class I alpha subunit complex. The latter associates
itself with transferrin receptor, downregulating iron uptake. Mutations
in HFE cause the protein to get stuck in the ER resulting in excess
transferrin-transferrin receptor interaction and subsequent iron
uptake into cells. The complete mechanism of the regulation of iron
uptake with HFE, however, remains somehow elusive.
As mentioned for the basic glucose transport
in intestinal enterocytes, ion gradients play an essential part
in the flow of metabolites. Prolonged flow will dissipate these
gradients making them less efficient and thus rebuilding these gradients
is essential. This is the function of pumps or ATPases
that use chemical energy released from ATP hydrolysis to push ions
against their gradient across cell membranes. Some pumps (F-type)
work in reverse and use proton flow to synthesize ATP. These ATP
synthases are essentially molecular motors coupling proton flow
to a rotational mechanism that affects the conformation of enzymatic
subunits in a cyclic way promoting condensation reactions between
ADP and inorganic phosphate. Pumps are estimated to consume up to
50% of all metabolic energy as ion gradients are important for transport
(flux coupling) and signal generation (action potentials and calcium
signaling). The primary energy form for all life comes from photosynthesis
and light independent electrogenic sources that couple electron
transport systems (e.g. mitochondria, chloroplasts, bacterial membranes)
to proton pumping. The F-type ATPsynthases convert the proton gradients
into ATP and ATP can be used to fuel all metabolic processes, including
Na, K, H, and Ca pumps (P-type ATPases).
Pumps are highly substrate specific and have a slow kinetic, compared
to carriers and channels. In addition to their selectivity, they
also display a tightly controlled stoichiometry when pumping more
than one ion species, usually in an antiport fashion (exchangers).
Thus, the Na/K-ATPases pumps 3 sodium ions for every two
potassium ions, while the K/H-pump exhibits a 1:1 stoichiometry.
The indicated number of ions are transported for every molecule
of ATP hydrolyzed. The hydrolysis is performed in two steps, where
the gamma phosphate is transferred to the pump (phosphorylation
step), and the phosphorylated enzyme eventually dephosphorylated
(completing the hydrolysis step). This two step mechanism where
the pump itself is temporarily phosphorylated appear to allow the
separation of the countertransport of the ions pumped across the
membrane. The dephosphorylated state binds sodium on the cytoplasmic
face, and phosphorylation releases the sodium ions from the pump
on the extracellular side of the membrane. The phosphorylated, sodium
free pump then binds two potassium ions on the outside. Dephosphorylation
of the pump transfers the potassium ion across the membrane and
releases them into the cytoplasmic compartment.
For all their importance in basic membrane energy metabolism, it
is not surprising that defects in pumps are associated to diseases.
Pernicious
anemia is a defect in vitamin B12 absorption. It is an autoimmune
disease of the stomach lining against the H/K-exchanger resulting
in the loss of chief and parietal cells. These cells are the main
lining cells and responsible to control pH and secretory function
of hormones and intrinsic
factor. The latter is a protein that binds cobalamine (Vitamin
B12) protecting it from the acidic condition of the stomach. The
H/K-exchanger can also serve as therapeutic target to treat peptic
ulcer. This ulcer is caused by the bacteria Helicobacter pylori
that thrives at low pH and grows in the gastric pit folds of
the stomach lining. Inhibiting the pump for several weeks will cause
elevated pH values that negatively affect growth of H.pylori.
Some pumps are specific for divalent cations and minor minerals.
Copper transport appears to be controlled by P-type ATPases. Defective
enzymes have been linked to inhibited copper uptake in Menkes
Syndrome and Wilson's
Disease resulting in copper accumulation and toxicity to the
liver and brain. Menkes Syndrome causes severe cerebral degeneration
and arterial changes, resulting in death in infancy.
An other class of pumps that relies on phosphorylation
from ATP hydrolysis are the ABC transporters (ATP cassette
binding proteins). ABC transporters have very diverse functions
and show a broad substrate range, unlike they highly specific F-
and P-type ATPases. They can transport ions, nucleotides, lipids,
peptides, and a plethora of organic molecules with and without charged
groups. This class of transporters has been first described for
P-glycoprotein and multi-drug resistance mechanisms. It is related
to bacterial transporters that play essential roles in metabolite
uptake like sugars and amino acids. Interestingly, ABC transporters
have conserved domains that are found in DNA binding proteins (associated
to nucleotide binding region, ATP cassette). Comparison of these
DNA binding proteins and their ligand binding properties (e.g. ligand
controlled transcription factors) with ABC transporters have been
used to create models of substrate transport in multi-drug resistance.
ABC
transporters have been implicated in diseases. Cystic
Fibrosis (CF) is an imbalance of chloride secretion in epithelial
cells where mutation in the cystic fibrosis transmembrane regulator
(CFTR) protein cause defective chloride transport across apical
cell membranes. Cystic fibrosis is characterized by a slow accumulation
of mucus and debris in the capillaries surrounded by the epithelia.
Low or defective chloride secretion reduces also water flow. The
water flow is necessary to clear capillaries from particles and
fibrils that may accumulate. The most common defect in the CFTR
transporter is related to a deletion mutation that causes a trafficking
defect and lack of transporter on the cell surface. Other mutations
cause defective regulation (ATP binding) or chloride conductance.
An other defect associated to ABC transporters is called sitosterolemia
and is caused by defective sterolins (ABC G5 and G8). Sterolins
function to expel plant sterols (e.g. sitosterol) in the intestine,
but also have a low affinity for cholesterol. As a result no plant
sterols and less than half of dietary cholesterol is absorbed. Defective
sterolins cause a loss of control of sterol secretion resulting
in unhindered absorption of plant sterols and dietary cholesterol.
Cholesterol absorption is easily prevented by omitting cholesterol
from the diet. The liver will be able to provide all necessary cholesterol
through de novo biosynthesis. The important endoplasmatic
reticulum enzyme HMG-CoA reductase is the major target of cholesterol
lowering drugs, the statins. A related cholesterol transport defect
causes Tangier
disease (TD), a genetic disorder of caused by ABC1 transporters
that is expressed in most cells and used to secrete excess cholesterol.
Bile production in liver depends on the secretion of many organic
molecules, some of which are negatively charged. ABC2 transporters
are selective for organic anions dubbed multi-specific organic anion
transporter (MOAT). Dubin-Johnson
syndrome is caused by mutations in the canalicular multispecific
organic anion transporter of liver hepathocytes resulting in conjugated
hyperbilirubinemia. The latter is a degradation intermediate of
heme molecules and will accumulate to toxic levels if the transporter
is defective.
Back
to Top
Metabolic disorders of membrane
lipids
Most membrane bound disorders are recognized as being the result
of faulty proteins. Lipids, however, play an important part in signaling
processes, membrane stability, and distribution of cell surface
receptors in polarized cells. Lipids are also found as anchors of
peripheral membrane proteins, many of which are involved in membrane
biosynthesis, transport, and signaling (e.g. G-proteins). Lipid
disorders are associated to both defective biosynthesis and faulty
degradation of specialized lipids. The latter is leading to the
accumulation of metabolic intermediates that cause toxic effects,
usually in neurons. Tay-Sachs
and Gaucher
disease are caused by defective degradation of gangliosides.
Enzyme replacement therapy became available as the first effective
treatment for Gaucher disease. The treatment consists of a modified
form of the glucocerebrosidase enzyme given intravenously. Some
lipids serve as anchors of membrane proteins. Hemoglobinuria
(blood in urine) is related to a defective synthesis of the glycosyl
phosphatidylinositol (GPI) anchor of an extra-cellular protein that
protects red blood cells from chemical damage. Excessive cell damage
leads to high concentration of globin in the urine. A defective
synthesis of protective myelin sheets around peripheral neurons
have been identified in Charcot-Marie-Tooth
(CMT) syndrome. CMT is a multifactorial
syndrome caused by different proteins and affecting different
peripheral neuronal processes. The underlying mechanism of the disease
is a dysfunction of the myelination of axonal extensions of neurons.
Lack of myelination causes a slow down of neuronal signaling (action
potential speed). Different proteins can be affected including the
gene encoding for the peripheral myelin protein-22 (PMP22), the
gene encoding myelin protein zero (MPZ, a sensorineural peripheral
polyneuropathy), the lamin A/C gene (a nuclear protein; mutations
cause a wide spectrum of neuromuscular and cardiac lipodystrophies,
with the functional consequence of this mutation seemingly associated
with a disorganization of the lamina), the gene encoding ganglioside-induced
differentiation-associated protein-1 (GDAP1), the small GTPase late
endosomal protein RAB7 (trafficking defects), the gene encoding
glycyl tRNA synthetase (GARS), and X-linked
Charcot-Marie-Tooth disease (CMTX) with proven connexin
32 (Cx32 or beta1) mutation associated with deafness. Sensorineural
deafness observed in this family suggests that Cx32 could play an
important role in the auditory pathway.
Lipids are also involved in mediating inflammatory responses during
infections and the coordination of energy homeostasis in the body.
Lipids such as prostaglandins and leukotrienes are
now recognized as signaling molecules that function as ligands for
membrane bound receptors (GPCRs) and thus their presence or absence
are inherently important for a healthy life. They are derived from
polyunsaturated fatty acids such as arachidonic acid and
eicosapentanoic acid. As signaling molecules, prostaglandin and
leukotriene derivatives may be well suited as drugs to treat diseases
by activating pathways that can compensate for loss of function.
A new glaucoma
drug activates an F2 receptor that stimulates extracellular matrix
metallo proteases (MMPs) that cause tissue degradation clearing
a pathway for fluid drainage and thus reducing built-up intraocular
pressure that over long period of times will damage the retinal
surface. Other glaucoma drugs are beta-adrenergic antagonists reducing
pressure build up.
Lipids Online - Educational
Resources in Atherosclerosis
Back
to Top
Gene therapy and drug
delivery
As became evident from the previous chapters, cell membranes are
veritable barriers to all sorts of molecules but contain specific
transport systems that are under cellular and physiological control.
Since both viruses and certain toxins use the intracellular machinery
for their replication or cytotoxic activity, respectively, they
need a cell entry pathway. To get into their host cells,
viruses and toxins bind to cell surface receptors inducing membrane
fusion or activating the endocytotic machinery, a physiological
up-take mechanism of cells for the import of large particles into
cells. These patho-physiological processes can be mimicked and exploited
for the targeted introduction of therapeutic drugs. The same mechanisms
are used for genetherapy applications, where healthy genes
are (re)introduced into cells of diseased tissues or organs. Three
basic mechanisms are most commonly used to develop systems for the
targeted delivery of macromolecules into cells: they include liposome
fusion, toxin transport, and viral uptake. The problem with toxins
and viruses using receptor mediated endocytosis is that this pathway
ends in intracellular organelles instead of in the cytoplasm. The
solution to this problem is to use various techniques to develop
immunotoxins, fusogenic peptides, or artificial viruses with the
ability to cross the membranes of these intracellular organelles.
Viral envelopes and bacterial toxins are examples of delivery mechanisms
that also provide specificity of interaction via cell surface receptor
binding. In addition to gene therapy and the introduction of nucleic
acids into cells, protein replacement therapy is of growing
interest and depends on the functional reconstitution of membrane
proteins. Liposome fusion can also be used to introduce either intracellular
proteins or cell surface receptors, where the membrane protein is
reconstituted into the liposome (vesicle) membrane prior to fusion.
Additional ligands can guide the liposomes to specific target cells.
Fusogenic proteins such as haemaglutinine in the liposome
membrane will increase the efficiency of liposome fusion with cell
membranes.
Viral vectors: Viral vectors for genetherapy include Adenovirus,
Hepatitis B (HBV), and Herpes Simplex 1 (HSV-1), all of which replicate
in the nucleus of human cells and insert their genetic material
into the genome of their host cells. Genetically modified viral
particles can thus be used to introduce a gene of interest into
a particular cell type, tissue, or organ.
Toxins: Two widely used toxins as vehicles for drug or DNA
transport into cells are diphtheria toxin (DT) and cholera toxin
(CT). Both toxins are composed of two subunits, a membrane transport
subunit and a cytotoxic subunit. The latter is transported across
lysosomal membranes triggered by acidic conditions into the cytoplasm
where it inhibits protein biosynthesis. Linking drug or DNA molecules
to the cytotoxic subunit may result in the import of chimeric molecules
into the cytoplasm of target cells.
Fusion process: A variety of self-assembling complexes exhibiting
specific binding properties can be used to import large quantities
of a drug or DNA of interest into cells via receptor mediated endocytosis.
Self-assembly systems include cationic (positively charged) liposomes,
lipoproteins, or other synthetic polymers coated with polylysine-conjugates
that promote receptor specific attachment to cell surfaces. The
presence of multiple positive charged surface groups promotes the
binding of negatively charged DNA molecules to these synthetic transport
vehicles. In all cases, the particle undergoes a fusion with the
targeted cell membrane releasing its contents into the cytoplasm
of the cell.
Peptides have been shown to be important as small molecules
interacting cell membranes. This interaction is mostly used as defense
mechanism leading to disruption of the attacked membrane and
the subsequent death of the cell or organisms. These peptides are
amphipathic forming large and unregulated pores in membranes depleting
ion gradients. Many naturally occurring ionophoric peptides have
been described. They function often as antibiotics and thus bioengineering
strategies are employed to mimic the function of these peptides
and find novel antibiotics that are able to overcome the
resistance many bacteria are evolving against common antibiotics
used in hospitals. Peptide nanotubes are designed based on cyclic
characteristics found in the naturally occurring antimicrobial Gramicidin
peptides. Because these cyclic peptides are designed to stack on
top of each other forming short tubes perforating cell membranes,
combinatorial rearrangements of peptide rings with differing amino
acid composition can quickly be screened to adapt antibiotics to
specific bacterial clones that emerge in hospitals and agricultural
settings.
Back
to Top
UCSD
Extension
MembraneHome
UCSD Home
H
o m e
Copyright © 2000-2013
Lukas K. Buehler
|